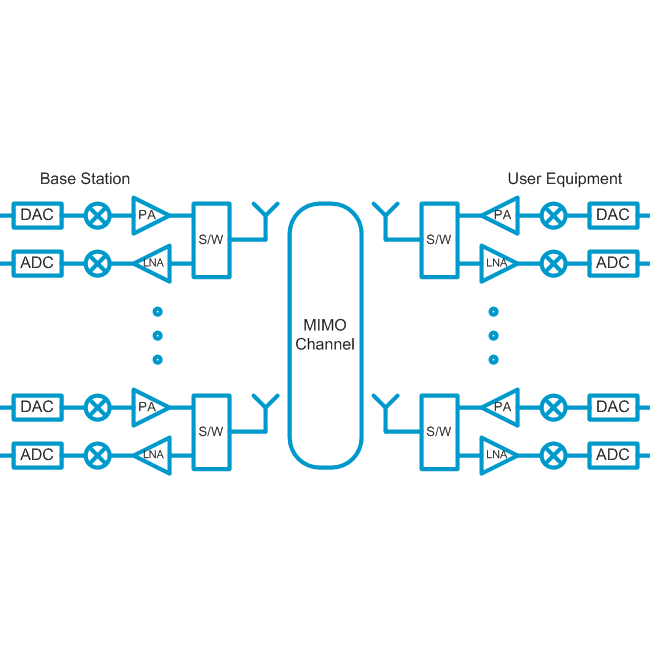
The complexity of the cellular infrastructure has evolved from 2G to LTE and now 5G. The expected 5G speeds reaching 1000x that of LTE will not only enhance existing telecom services, but also lay a new infrastructure for emerging applications such as virtual/augmented reality, self-driving cars, the internet of things (IoT) and wearable and implantable devices [1-2].
The 5G architecture will employ multiple input multiple output (MIMO) and beamforming technology to direct signal power for increased over-the-air data rates. A number of demonstration 5G systems achieving more than 3Gb/s have been published in the literature [2-3]. These MIMO architectures will stimulate new design goals on the size and capabilities of the RF transceiver hardware. A block diagram of a digital beamforming 5G massive MIMO architecture is shown in Fig. 1.
Several key directions for 5G have emerged. First, the critical allocation of spectrum will dictate the design and implementation of transceiver hardware. Due to the highly congested sub-6-GHz cellular bands, mm-Wave frequencies are necessary for achieving the desired low-latency, high speed transmission. The FCC has approved of several bands for leading cellular carriers including 28GHz (Verizon, AT&T, T-Mobile), 37GHz and 39GHz (T-Mobile) [4]. Initial development at 28GHz is the most likely but still has significant challenges. While full mm-wave 5G infrastructure is being developed, carriers will first implement sub-6GHz 5G systems employing many of the same MIMO beamforming techniques but at lower, more technologically accessible frequencies. A number of sub-6GHz 5G MIMO systems have been demonstrated at 3.3-4.2 GHz [5].
Massive MIMO beamforming will require a multiplicity of RF circuitry for each antenna element in the phased-array transceiver system. Therefore, size, cost and power density are crucial figures of merit for both the base station and handset architectures. Analog, digital and hybrid beamforming techniques are under consideration. A multiplicity of RF transmit and receive chains will be required as shown in Fig. 1.

References
- Ericsson, Stockholm, Sweden, “5G systems,” 2017. [Online]. Available: https://www.ericsson.com/res/docs/whitepapers/wp-5g-systems.pdf
- Gozalvez, “Samsung Electronics Sets 5G Speed Record at 7.5 Gb/s,” IEEE Vehicular Technology Magazine, Feb 2015, pp. 12-16.
- Sadhu, Y. Tousi, J. Hallin et al., “A 28GHz 32-Element Phased-Array Transceiver IC with Concurrent Dual Polarized Beams and 1.4 Degree Beam-Steering Resolution for 5G Communication,” 2017 IEEE Solid-State Circuits Conference, Feb 2017.
- 5gamericas, Bellevue, WA, “5G Spectrum Recommendations,” 2015. [Online]. Available: http://www.5gamericas.org/files/6514/3930/9262/4G_Americas_5G_Spectrum_Recommendations_White_Paper.pdf
- Goovaerts, “Intel Unveils 5G Modem with Support for Sub-6 GHz, 28 GHz at CES,” [Online]. Available: https://www.wirelessweek.com/news/2017/01/intel-unveils-5g-modem-support-sub-6-ghz-28-ghz-ces
- Wolfspeed, “GaN HEMT MMICs,” Accessed April 2017. [Online]. Available: http://www.wolfspeed.com/rf/foundry-services/gan-hemt-mmic
- Global Comm. Semi., “GaAs & GaN RF Technologies,” Accessed April 2017. [Online]. Available: http://www.gcsincorp.com/dedicated_pure-play_wafer_foundry/GaAs%20&%20GaN%20RF%20Technologies.php
- Northrop Grumman Corp., “Gallium Nitride (GaN) HEMT for High-Power, High-Frequency Electronics,” Accessed April 2017. [Online]. Available: http://www.northropgrumman.com/BusinessVentures/Microelectronics/Products/Pages/Gallium-Nitride-(GaN)-HEMT.aspx
- Ommic, “Technology,” Accessed April 2017. [Online]. Available: http://www.ommic.com/site/tech-2
- Qorvo, “Gallium Nitride (GaN), GaN: The Industry’s Hot Technology,” Accessed April 2017. [Online]. Available: http://www.qorvo.com/innovation/technology/gan
- National Research Council Canada, “GaN Design Kits,” Accessed April 2017. [Online]. Available: http://www.nrc-cnrc.gc.ca/eng/solutions/advisory/gan_design_kit/index.html
- UMS, “Foundry Services,” Accessed April 2017. [Online]. Available: http://www.ums-gaas.com/telechargement/1606_Brochure_Foundry_2016.pdf
- S. Yuk, G. R. Branner, and D. J. McQuate, “A Wideband, Multiharmonic Empirical Large-Signal Model for High-Power GaN HEMTs With Self-Heating and Charge-Trapping Effects, IEEE Trans. MTT, vol. 57, no. 12, pp. 3322-3332, Dec. 2009.
- -S. Noh, Y.-H. Choi, I. Yom, “Ka-band GaN power amplifier MMIC chipset for satellite and 5G cellular communications,” 2015 IEEE 4th Asia-Pacific Conference on Antennas and Propagation (APCAP), June 2015.
- Northrop Grumman, West Falls Church, VA , “APN228 27-31 GHz GaN Power Amplifier Datasheet Revision: April 2015,” Apr. 2015. [Online]. Available: http://www.northropgrumman.com/BusinessVentures/Microelectronics/Products/Documents/pageDocs/APN228rev0415.pdf
- Micovic; D. F. Brown; D. Regan; J. Wong; Y. Tang; F. Herrault; D. Santos; S. D. Burnham; J. Tai; E. Prophet; I. Khalaf; C. McGuire; H. Bracamontes; H. Fung; A. K. Kurdoghlian; A. Schmitz, “High frequency GaN HEMTs for RF MMIC applications,” 2016 IEEE International Electron Devices Meeting (IEDM), Dec. 2016.
- Kallfass, G. Eren, R. Weber, S. Wagner, D. Schwantuschke, R. Quay and O. Ambacher, “High linearity active GaN-HEMT down-converter MMIC for E-band radar applications,” 2014 Euro. Microw. Int. Circ. Conf., Oct. 2014, pp. 128-131.
- M-N. Do, M. Seelmann-Eggebert, R. Quay, D. Langrez, J-L. Cazaux, “AlGaN/GaN mixer MMICs, and RF front-end receivers for C-, Ku-, and Ka-band space applications,” 2010 Euro. Microw. Int. Circ. Conf., Sept. 2010, pp. 57-60.
- van Heijningen, J.A. Hoogland, A.P. de Hek, F.E. van Vliet, “6-12 GHz Double-Balanced Image-Reject Mixer MMIC in 0.25um AlGaN/GaN Technology,” Proc. Of 9th Euro. Microw. Int. Cir. Conf., Oct. 2014.
- Henderson, E. Camargo, Microwave Mixer Technology and Applications, Artech House, Jul. 2013.
- -Y. Wong, W. Chen, Q. Zhou and K. J. Chen, “Zero-Bias Mixer Based on AlGaN/GaN Lateral Field-Effect Diodes for High-Temperature Wireless Sensor and RFID Applications,” IEEE. Trans. On Electron Dev., Vol. 56, No. 12, Dec. 2009, pp. 2888-2894.
- Polli, M. Palomba, S. Colangeli, M. Vittori, E. Limiti, “High power-handling GaN switch for S-band applications,” Integrated Nonlinear Microw. and Millimetre-Wave Circuits Workshop, Apr. 20-21, 2017.
- Bunz, R. Reber, P. Schuh, M. Oppermann, “High power broadband GaN switch MMICs,” Microwave Integrated Circuits Conference (EuMIC), Sept 2015.
- Cory and D. Fryklund, “Solid State RF/Microwave Switch Technology: Part 2,” Accessed June 2017. [Online]. Available: http://www.skyworksinc.com/downloads/press_room/published_articles/MPD_062009.pdf
- Analog Devices, “HMC547LC3 GaAs SPDT Non-reflective Switch, DC-28GHz,” Accessed June 2017. [Online]. Available: http://www.analog.com/media/en/technical-documentation/data-sheets/hmc547lc3.pdf
- Peregrine Semiconductor, “PE42525 UltraCMOS SPDT RF Switch, 9kHz-60GHz,” Accessed June 2017. [Online]. Available: http://www.psemi.com/pdf/datasheets/pe42525ds.pdf
- S. Yuk, G. R. Branner, and C. Wong, “High Power, High Conversion Gain Frequency Doublers using SiC MESFETs and AlGaN/GaN HEMTs,” in IEEE MTT-S Int. Microw. Symp. Dig., pp. 1008-1011, May 2010.